Novel applications of proton therapy in breast carcinoma
Introduction
Breast cancer is the most common malignancy diagnosed among females in the United States, with an estimated incidence of 231,840 cases in 2015 (1). Long-term follow-up of the National Surgical Adjuvant Breast and Bowel Project (NSABP) trials (2) and EBCTCG meta-analyses (3,4) demonstrated that the prevention of local recurrence after surgery for breast cancer translates into reduced breast cancer mortality. Today, radiation therapy remains a vital component of the curative multimodality therapy for many women with localized breast cancer. Aside from select older women with small, hormone receptor positive breast cancer, most women who opt for breast conservation therapy are recommended adjuvant radiation as part of breast conserving therapy. In more advanced disease, many women require post-mastectomy radiation and/or regional node irradiation.
The successes of prolonging the lifespan of breast cancer patients via locoregional recurrence prevention have also sharpened the awareness of long-term costs of such efforts. With improvements in surgery, systemic therapy, and radiation, the majority of women diagnosed with breast cancer will be cured of their disease and live for decades. With an increasing number of breast cancer survivors, late treatment related toxicities have gained more attention lately. The original Early Breast Cancer Trialist Group (EBCTG) analysis showed that the improved disease control benefits of radiation were offset by radiation related morbidity and mortality. Proton therapy offers an opportunity to continue to improve the therapeutic ratio for breast cancer patients by targeting tissue at risk and optimizing tumor control, while simultaneously maximally sparing non-target tissue and reducing treatment morbidity. This review will focus on emerging practices and techniques for the use of proton therapy for both early and advanced stage breast cancer.
Partial breast irradiation (PBI)
The central rationale of PBI is based on observations of early breast conservation trials comparing surgery with or without whole breast radiation therapy. In these trials, the predominant site of local recurrence was in close proximity to the original site of the tumor. Furthermore, the rates of failure at locations at a considerable distance from the site of the tumor were not different between those patients who did or did not receive radiation therapy to the breast (2,5,6). These observations led to the hypothesis that limiting the size of the radiation target to only the tissue at highest risk of local recurrence would provide equivalent local control for patients.
PBI offers several potential advantages to conventional whole breast radiation. A smaller target allows for larger doses per fraction and shorter overall treatment time (accelerated hypofractionation). Fewer, larger treatments are more convenient for patients and more cost effective for the healthcare system. Decreasing the amount of irradiated tissue would be expected to mitigate long-term cosmetic adverse effects. Hypofractionation is also based upon sound radiobiological principles that suggest a low the alpha beta ratio for breast cancer (7,8).
There are many well accepted forms of PBI delivery including IORT, interstitial brachytherapy, intracavitary brachytherapy, and external beam radiation (EBRT). Currently, EBRT seems to be the most commonly used form of PBI as seen by the enrollment patterns in the interim toxicity report of the NSABP B-39 trial which randomized women with early stage breast cancer ≤3.0 cm with negative margins, and ≤3 positive nodes to partial or whole breast irradiation (9). Early enthusiasm for external beam PBI was strengthened by promising early results reported by the groups at New York University and the William Beaumont Hospital (10-14). These early efforts led to development, implementation, and successful accrual of large phase III randomized trials, including the Randomized Trial of Accelerated Partial Breast Irradiation (RAPID) and NSABP B-39 trials. Even as mature data from these trials are pending, the use of this approach continues to increase outside the confines of a clinical trial. This prompted the American Society of Radiation Oncology (ASTRO) to issue a consensus statement to help guide the selection of suitable patients for accelerated PBI as practitioners await these data (15). Most study results surrounding recurrence rates have yet to mature and provide definitive guidance regarding the efficacy of PBI.
There are several studies with reasonable follow-up investigating the effect of PBI on long-term cosmesis. Results of single arm studies using intensity modulated radiation therapy have generally been favorable. Chen et al. reported a good/excellent cosmesis rating in 89% at four years among 94 patients treated with 38.5 Gy in 10 fractions (16). Thirty-six patients were treated to 38 Gy in 10 fractions by the group at the Baptist Hospital in Miami, FL, and 94% reported an overall cosmesis of excellent or good after a median follow up of nearly 45 months (17). A prospective Phase II trial reported by Lei et al. showed excellent cosmesis in 88.2% of patients after treatment with 38.5 Gy in 10 fractions to a volumetric expansion of 2 cm on the lumpectomy cavity (18). The group at the Medical College of Wisconsin similarly reported that 95% of patients had good to excellent cosmesis after treatment to an equivalent dose and volume (19).
In contrast, the University of Michigan reported unfavorable outcomes for their trial of APBI using IMRT and active breathing control, which was terminated early due to unacceptable cosmesis at an interim follow up period (20). Publication of the interim cosmetic results of the RAPID trial added to the cause for concern; after a median follow up of 36 months, those patients undergoing ABPI has significantly worse cosmesis compared with those patients undergoing WBI. The inferior cosmesis was seen across patient, nurse, and physician reported assessments (21).
The decline in cosmesis was further observed in the latest analysis of the University of Michigan study (22). In attempting to identify factors associated with such high rates of poor cosmesis, the authors discovered statistically significant differences in the average whole breast volumes receiving the prescribed dose between those patients with an excellent or good cosmetic outcome, and those with a fair or poor outcome. As noted by the authors, the association between dose volume parameters and toxicity had been previously reported in early toxicity analyses of NSABP B39 (23,24). These observations indicate that cosmesis is correlated to the volume of non-target breast tissue receiving both prescription dose and lower dose level parameters, including V5, V20, V50 and V80. These data indicate that limiting the volume receiving not only prescription dose, but other dose levels as well, is paramount to the preservation of favorable cosmetic outcomes. The optimal fractionation scheme for EBRT PBI is also not defined and it may be that once daily PBI over 2 weeks would be better tolerated than twice daily over one week (25).
There are a number of evolving techniques that have shown promise in limiting morbidity and sparing healthy tissues with the use of PBI, including preoperative treatment (26), single fraction therapy delivered in the preoperative setting(27), and stereotactic therapy using Gamma emitting sources (28,29). Several investigators have also explored the potential advantages of proton therapy in the PBI setting. Although some of the studies involving protons have found statistically significant reductions in terms of doses to the heart and lung, these likely represent clinically insignificant differences, as heart and lung doses are typically minimal even with photon based PBI techniques. Data from Beaumont (utilizing 4 or 5 field 3D conformal photons in the supine position), New York University (tangential photons in the prone position) and Massachusetts General Hospital-Beth Israel Deaconess Medical Center (passive scattering protons using 1–3 fields) show ipsilateral lung V20s ranging from 0% to 4%, V10 from 0–9%, and V5 From 0–16%. Heart V20s, V10s and V5s were all 0% (13,30,31). Thus, while there are certainly exceptions, we believe it is hard to justify the use of proton therapy as a way to further reduce heart and lung dose for most patients routinely, although there may be select cases with challenging anatomy where protons might be helpful.
However, where protons might make a meaningful difference when used for PBI is in their ability to limit dose to the non-target breast tissues. These include the skin and chest wall, which are at risk for late toxicity after balloon based brachytherapy (32,33), and of the uninvolved lateral, deep, superior, and inferior breast tissue in the beam path. It is now widely hypothesized that the sparing of non-target breast tissue is critical in ensuring favorable cosmesis after ABPI. Studies comparing techniques for ABPI suggest that limiting this dose can be difficult with traditional photon techniques. The eligibility requirement for the B39 trial was less than 50% of the non-target breast receiving 50% of the prescription dose. It is often challenging to meet this requirement with 3DCRT in women with large seromas and small breasts. A dosimetric study comparing external beam to brachytherapy found that the use of 3DCRT resulted in higher percentage of the breast receiving 100% and 50% of the prescribed dose compared to interstitial and MammoSite techniques (34). A dosimetric investigation of external beam techniques for ABPI found that 3DCRT provided the least favorable profile of non-PTV breast volume receiving 50% of the prescribed dose (mean 40.9%) compared with IMRT (33.3%), and Tomotherapy (22.8%). The technique with the most favorable sparing of the breast was proton therapy (16.5%) (35). Other studies, including the series of 25 patients treated with twice daily passive scattering proton beam PBI published by Taghian et al. (31), reported the non-target breast V50 dose was significantly smaller with protons (23%) compared to other photon techniques (39–42%).
Although limiting dose to the non-target breast is important for reducing fibrosis and optimizing overall cosmesis, early clinical experience shows that skin toxicity and telangiectasias may result if proton therapy is delivered by a single beam. An early series from the Massachusetts General Hospital reported early outcomes and toxicity among twenty early stage patients. Physicians used one to three beams to treat to 32 Gy [relative biological effectiveness (RBE)] in 4 Gy (RBE) per fraction twice daily. For patients treated with protons, a single field was treated each fraction—even patients with 2–3 field plans had only a single field treated each day due to machine time availability. There was an initial decline of cosmesis after therapy, although cosmesis later improved and 100% of patients reported good or excellent cosmesis at 12 months (36). MGH expanded on their initial series with a prospective clinical trial of 98 patients treated with protons, photons, or mixed photons/electrons. After a median follow up of 82.5 months, patients treated with protons were found to have a higher rate of telangiectasia (69% vs. 16%), pigmentation changes (54% vs. 22%), and worse rates of good or excellent cosmesis (62% vs. 94%) than their counterparts treated with photons (although there were no differences in patient reported outcomes between the cohorts) (37). In support of this finding, Chang et al. reported that treatment with two proton fields resulted in a higher percentage of patients with good or excellent cosmetic outcomes compared to patients treated with a single field (38). A larger phase II trial of 90 patients from Loma Linda used 2–4 beams to deliver 40 Gy (RBE) in 10 fractions (39). Five-year follow up results showed good to excellent cosmesis in 90% of patients (40).
Multi-beam treatment when using double scattering protons is therefore recommended in the treatment of breast cancer with protons. Today, most institutions use at least two fields per day. Multiple beam angles also allow for generation of more robust plans, given the relative mobility, deformability and setup uncertainty of the breast as a target. Going forward, the use of pencil beam scanning (PBS) will allow for modulation of the entrance dose allowing for skin sparing and further reduction of dose to non-target tissue. Further study is needed to optimize and validate this technology as a way to improve outcomes for women compared with other modern PBI techniques.
In summary, the final results of the RAPID and NSABP B39 studies are needed to define the role PBI in the treatment of breast cancer. Should ABPI prove to offer a clinically meaningful benefit to patients, proton therapy could offer significant advantages over both photon and brachytherapy based techniques. By reducing the dose to non-target breast tissue, proton therapy might offer the ideal dose distribution for APBI and this may result in improved cosmesis, especially when multiple fields and PBS are used. However, further study and longer follow up is needed to determine if such a benefit exists.
Whole breast/chest wall and regional nodal irradiation
Decreasing locoregional recurrence with adjuvant radiation therapy contributes to improved breast cancer specific survival after breast conserving surgery (4) and improved overall survival after mastectomy (41-43). For those patients at a high risk for nodal failure, inclusion of the axillary, supraclavicular, and internal mammary lymph node chains also improves survival. It is important to remember that the landmark post-mastectomy radiotherapy (PMRT) trials employed comprehensive regional nodal irradiation (RNI), including the internal mammary chain. However, targeting these lymph node groups often leads to the irradiation of large amounts of normal tissue and can increase the risk of treatment morbidity. The selection of patients for comprehensive nodal irradiation and the extent of target delineation have become subjects of intense debate.
The recent publication of two landmark trials in the New England Journal of Medicine has confirmed the importance of RNI for women with relatively early stage breast cancer (high risk node negative and women with 1–3 positive lymph nodes). The MA.20 trial assigned 1,832 women with 1–3 involved lymph nodes or high-risk node negative (defined as a primary tumor >5 cm, or tumor >2 cm with less than 10 axillary lymph nodes removed and at least 1 of the following: grade 3 histology, estrogen receptor negativity, or lymphovascular invasion) (10%) breast cancer to adjuvant whole breast irradiation with or without nodal irradiation to the axillary, supraclavicular, and internal mammary lymph nodes. Radiation therapy was delivered with conventional techniques including opposed tangents with a nondivergent anteriorly oriented supraclavicular field. The internal mammary lymph nodes were covered with either wide tangents or a mixed photon-electron field angled to match the tangents. Isolated loco-regional recurrence free survival, disease free survival, and distant metastasis free survival were improved with RNI (44).
The European Organisation for Research and Treatment of Cancer (EORTC) 22922 trial showed similar findings. This study randomized 4,004 patients with either medial breast tumors (regardless of axillary involvement) or node positive disease to undergo whole breast or chest wall irradiation with or without medial supraclavicular and internal mammary nodal targeting using techniques similar to the MA.20 trial, although the wide tangent technique to cover the internal mammary lymph nodes was discouraged in this trial. With a median follow up of 10.9 years, there was a disease-free survival benefit and a reduction in breast cancer mortality with regional nodal irradiation. There was also a trend towards an overall survival benefit (45). It should be noted that the absolute benefit of nodal irradiation would likely have been greater if a higher risk group of patients were investigated. For example, 60% of patients in the EORTC trial had T1 tumors and 45% were node negative. This is a lower risk subset of patients than are typically considered for internal mammary irradiation in the United States. Nevertheless, the study still demonstrated a significant improvement in meaningful endpoints including distant recurrence rates.
Although nodal radiation improves disease outcomes, targeting these nodal groups comes with a price. Nodal irradiation has been associated with an increased risk of radiation pneumonitis, lymphedema, and brachial plexopathy. The anatomical position of the internal mammary lymph nodes makes treatment particularly challenging. Perhaps most significant is the risk of coronary artery disease, myocardial infarction, or cardiac death (46-48). While this risk is greatest in those patients with left sided tumors, patients with right-sided tumors undergoing internal mammary directed treatment can still receive significant doses especially to the right coronary arteries.
The optimal coverage of target tissue in locally advanced breast cancer is often compromised by avoidance of organs at risk with traditional techniques. Although minimizing the volume of the heart receiving high doses can be relatively straightforward, there appears to be a dose-dependent association between ischemic heart disease and even low doses to the heart, with no safe threshold below which there is no increase in morbidity (49). A number of different cardiac-sparing techniques have been described by investigators (50,51). Most commonly 3DCRT is used with 3, 4 or 5 field techniques. However, a publication from MD Anderson showed that commonly used radiation fields do not offer adequate coverage of the chest wall or regional nodes in most cases (52). For high risk patients where more complete target coverage is needed, multifield intensity modulated radiation therapy can be used, however, the multiple beam angles often result in a higher integral dose resulting in a large volume of the cardiopulmonary structures receiving low doses when using IMRT (51). The significance of the low dose exposure is not well characterized. In modern practice, there is a delicate balance between target coverage and normal tissue sparing using photon-based techniques.
There are now at least six comparative treatment planning publications demonstrating that proton therapy improves target coverage and minimizes dose to organs at risk compared to 3D conformal photons or IMRT/volumetric arc therapy (VMAT) (53-59). Five of these studies focus on regional node irradiation in addition to the breast/chest wall. Mast et al. examined the benefit of protons when treating the left breast alone. Dosimetric comparisons regarding organs at risk are summarized in Table 1. Across all studies, proton therapy significantly reduced dose to cardiopulmonary structures and the contralateral breast despite equal or better target coverage with protons. The publication by Ares et al. highlighted the superiority of proton therapy when complex target coverage is needed; although proton therapy only offered a modest benefit when treating the breast and supraclavicular lymph nodes, the greatest benefit was clearly when the internal mammary lymph node chain was included in the target volume (53).
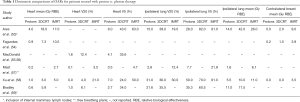
Full table
Based on the dosimetric advantages of proton therapy, several proton centers initiated prospective phase I/II single arm prospective trials in women with high-risk disease requiring comprehensive nodal irradiation and/or with unfavorable cardiac anatomy. Early clinical experience using passive scattering proton therapy has been reported by three groups. At Massachusetts General Hospital, 12 patients were treated with postmastectomy proton therapy with excellent target coverage, excellent organ-at-risk sparing, and no cases of grade 3 or 4 skin toxicity (56). The average mean heart dose was 0.44 (range, 0.1–1.2) Gy, the average mean lung dose was 6 (range, 2.4–10.1) Gy, and the average lung V20 was 12.7% (range, 4.4–22.1%). A series from Memorial Sloan Kettering Cancer Center/Princeton Radiation Oncology reported on 30 patients, including four treated with breast conserving surgery, and demonstrated favorable coverage of the targets which included axillary, supraclavicular, and, in 93% of cases, the internal mammary lymph nodes (60). The mean and low dose parameters delivered to the heart and lungs were low and consistent with the previously published dosimetric studies, with a median mean heart dose of 1 (range, 0.01–3.20) Gy and ipsilateral lung V20 of 16.5% (range, 0.14–13.2%). Toxicities were mild. The rate of grade 2 dermatitis was 71.4%, and the rate of moist desquamation was 28.6%. There were no grade 3 skin toxicities. One patient experienced a grade 3 reconstructive complication. Figure 1 demonstrates a typical course of skin reaction for patients undergoing both post-mastectomy and post-lumpectomy proton therapy with uniform scanning proton therapy. Bradley et al. recently reported the results of a prospective feasibility study at UFPTI using passive scattering protons for 10 breast cancer patients requiring comprehensive nodal irradiation. Doses to the heart and lung were comparable to those reported by MGH and MSK/Procure. Acute toxicity was also similar to previous photon and proton series.
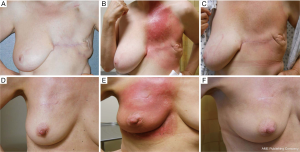
Acute toxicity: skin dermatitis and desquamation
The rates of acute skin toxicities in the MSK/Procure series were similar to what would be expected for patients receiving PMRT with photon irradiation using bolus. To confirm this finding, doses to the surface of the skin were measured by optically stimulated luminescent dosimeters (OSLDs) with passive scattering proton therapy, photon therapy, and photon therapy with bolus among 14 patients undergoing post-operative EBRT for breast cancer (four patients treated with photons and bolus, four post lumpectomy patients treated with photons, and six patients treated with protons). As shown in Figure 2, while there is significant skin sparing with photons without bolus, there were no significant differences between patients treated with photons + bolus and passive scattering protons (61). Thus, it is not surprising that skin toxicity seems comparable to historical reports of PMRT. In the post lumpectomy setting, protons would be expected to have higher skin toxicity and perhaps compromised cosmesis compared to photon tangent fields because of the higher skin dose.
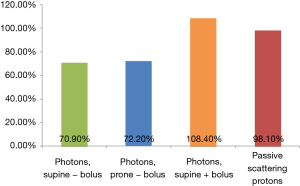
Late toxicity: cardiac disease
Heart disease continues to be the leading cause of death in the United States (62). Prevention of radiation induced coronary artery disease with adequate cardiopulmonary tissue sparing has a widespread and significant potential benefit. The encouraging early clinical results of proton therapy for breast cancer have aided in the development of the RADCOMP Trial, a pragmatic, randomized control trial of protons vs. photons for non-metastatic breast cancer in women requiring treatment of the internal mammary lymph nodes, with the primary endpoint of reduction in cardiac events at 10 years, activated in the spring of 2016 and with approximately 30 patients accrued as of July 2016.
Since the results of this trial and others will take years to mature, the long-term cardiac benefits will not be available for years. Efforts are underway to identify surrogate markers that accurately predict for subsequent cardiotoxicity. These surrogates could provide immediate feedback and insight into the clinical benefit of protons, should any such benefit exist. These surrogates would also allow high-risk patients to be identified for early intervention. Candidate serum biomarkers of cardiotoxicity include cardiac troponins, natriuretic peptides, heart-type fatty acid binding protein, glycogen phosphorylase isoenzyme BB, C-reactive protein, myeloperoxidase, and nitric oxide. Each of these markers has been studied, but results have been mixed and determining clinical utility has remained elusive, as reviewed by Tian et al. (63). A promising alternative is to combine serum biomarker data with cardiac imaging as analyzed by Sawaya et al. (64). The authors found that longitudinal myocardial strain and ultrasensitive troponin I was highly correlated with the subsequent development of cardiotoxicity among breast cancer patients treated with anthracyclines, taxanes, and trastuzumab. Similar correlation of radiation-induced changes in strain echo with clinical dysfunction does not exist. However, Erven et al. have published that patients with left-sided tumors receiving photon radiation with mean heart doses in the 5 Gy range, do have significant decreases in longitudinal strain and strain rate (used as markers of functionality in this study) as assessed by strain rate echocardiogram after RT (65). Unpublished data on 30 patients treated with proton therapy on the MGH protocol did not have changes compared to baseline after undergoing proton therapy (66). More work is needed to better define the ability of strain echo to detect subclinical radiation induced injury. Other modalities used to assess radiation induced damage include single-photon emission computed tomography (SPECT) (67,68) and cardiac MRI (69,70), although data is limited to date.
Technical considerations
Target delineation
Contouring of the CTV for protons and photons for the clinician should be identical since the CTV defines a volume at risk for harboring microscopic disease, regardless of treatment modality. However, contouring accuracy is much more important with highly conformal forms of radiation such as proton therapy (given the steep dose gradients). If an area is not contoured in the CTV, it will almost definitely not receive a meaningful dose. This is in contrast to 3DCRT and even IMRT where there is much more incidental dose to areas, making contouring less critical. At our institutions, contouring of clinical target volumes and normal structures is in line with the RADCOMP contouring guideline and follows the RTOG atlas (71) with a few important exceptions. The deep supraclavicular fossa (SCV) is a known area at risk (72,73) and receives a substantial incidental dose if the supraclavicular lymph nodes are treated with an oblique photon field. However, contouring the SCV lymph nodes according to the RTOG atlas when treating with proton therapy results in significant underdosing to this area (74). Therefore, the authors generally extend this portion of the CTV posteriorly to encompass the entirety of the deep SCV.
There is debate over the appropriate use of PTV margins for setup uncertainty and motion for breast cancer. A recent pattern of care survey (RADCOMP unpublished) showed that there is still variability regarding the use of PTV margins for breast cancer. In New Jersey, we tend to use a 7 mm margin medially and laterally but not posteriorly. Distal margins are set to account for proton range uncertainties. In addition, we do not add the margin medially in the supraclavicular region to avoid extending the PTV into the esophagus; when we did this originally, we found higher rates of esophagitis in our early clinical experience as would be expected if the PTV overlapped with the esophagus. Without a medial PTV margin, we are able to cover the CTV and limit the esophagus max dose to 40 Gy and have low rates of esophagitis (60).
The RTOG atlas for node positive patients includes the intercostal muscles and ribs, however, this is a low risk area and not felt to be at high risk for microscopic disease. Moreover, the lung chest wall interface represents a steep gradient of electron density and is less than ideal as a proton stopping structure. Our practice has been to exclude the intercostals and ribs; this is also consistent with the RADCOMP contouring guidelines. Excluding the ribs/intercostals allows the end of range to fall into the relatively homogenous and non-vulnerable tissue of the ribs and intercostal muscles. This creates a dose gradient along the ribs/intercostals with proton therapy. To date, there have been no recurrences in that area but this will need longer follow-up to verify that this does not compromise outcomes.
An additional concern is the higher RBE of protons compared to photons. RBE is the ratio of doses of two different types of ionizing radiation that produce an equivalent biological effect. Studies investigating the RBE of protons, mostly using clonogenic survival assays and measuring from within the center of the Bragg peak, have concluded that the RBE is roughly 1.1 (75,76). To date there have not been any clinical reports that suggest that this number is significantly inaccurate. However, there is a growing body of literature that suggests an enhancement of the RBE at the end of range (77-86). With an en face beam arrangement used in the treatment of breast cancer, the end of range is along the anterior ribs, and might be delivering a higher than anticipated biological dose to this area. Planning and delivery techniques, such as the use of multiple beam angles to feather hot spots and PBS with distal edge tracking, could mitigate this effect. To date, rib fractures have not been reported in our experience but close follow up is warranted to monitor for this toxicity.
Set up and delivery
At our institutions, patients are simulated with a CT scan with the arms usually abducted above the head using a custom mold (alpha cradle, breast board), although it is also possible to treat patients with the arms down. There is no actual immobilization of the breast. A chinstrap and shoulder pulls are often used. Proton therapy is typically delivered with 2 matching anterior oblique en face fields to cover the full length of the field. Given the limitation in field size with uniform scanning and passive scattering, matching fields are typically needed to cover the chest wall and supraclavicular nodes. The match line is feathered; specifically, two anterior oblique fields are matched at the chest wall/SCV junction, and an additional two anterior superior oblique fields with a match line at least a centimeter away from the anterior oblique field match line are used. The match line can be feathered daily with two fields treated per day or both sets of matched fields can be treated each day (four fields). A typical beam arrangement is shown in Figure 3.
In general, 45–50 Gy (RBE) is prescribed to the chest wall and regional nodes in 1.8–2.0 Gy per fraction. Set up accuracy is confirmed with daily X-ray verification of the isocenter based on bony anatomy. At some institutions, daily 2dKV imaging coupled with surface imaging is also used for set up.
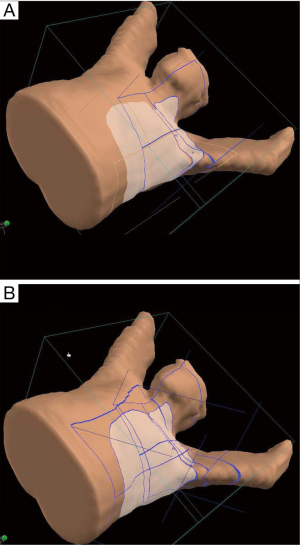
Most of the clinical experience has been with passive scattering and uniform scanning (56,59,60), although MGH has also reported on a PBS technique (87), and we anticipate PBS to be used with increasing frequency in the future. PBS allows for treatment with a single field (avoiding match lines, feathering, hot/cold spots), a faster treatment delivery, and some skin sparing. Unlike photons, protons do not have a dose build up at the skin surface and for superficial targets (such as the breast/chest wall), the skin usually receives full dose with scattered protons. PBS allows for some dose control of the proximal beam and some degree of skin sparing. PBS also reduces scatter and neutron dose to the skin by eliminating the need for compensators and apertures. Even with scattered protons, dose to the skin and rates of dermatitis were similar to that with photons with bolus (as described above), but PBS should further improve skin toxicity.
Planning and uncertainty mitigation
Proton therapy may be particularly sensitive to inter- and intrafraction uncertainty. The source of uncertainty is related both to intrafraction motion and set up variability. Specifically for breast cancer, uncertainty is also related to the extent of the initial surgery. While there is very little uncertainty for post mastectomy patients (both those with an unreconstructed chest wall and those with implant reconstruction), mobile breast tissue (for those patients with an intact breast) is a significant source of set up variability. Treatment related edema may also require adaptive planning. In our initial experience, only small breasted patients with minimal interfraction mobility were candidates for proton therapy after lumpectomy. Available options to confirm accurate set up include low dose verification CTs at prespecified points during treatment, and the use of optical surface tracking technologies prior to each fraction which have made treatment of intact breasts more feasible. While PBS does address some issues of range uncertainty, the fact that the timing of the delivery of the pencil beam spots and energy specific layers are similar in time scale to patient respiration could lead to spot scanning/respiratory motion interplay and result in potential underdosing or overdosing of target. This is of particular concern when using high doses per fraction, as in PBI. Treatment with deep inspiration breath hold or respiratory gating is an option to mitigate target motion effects. However, these techniques require a relatively constant delivery of beam so as to minimize patient breath hold times and treatment times, and may not be feasible for large centers that must share the beam among several high volume rooms.
Another potential challenge for the delivery of breast cancer patients is the increasing use of immediate tissue expander reconstruction. The metal port in the tissue expander placed after breast reconstruction perturbs the proton dose distribution. Large gradients of proton energy loss created by the variability of the stopping powers of the materials used in the tissue expanders creates an unacceptable dose profile. With apreture/compensator techniques, anterior beams are used and a cold spot is created behind the metallic port. The alternative is to increase the beam range to compensate for the increased density through the metal, but at the expense of more dose delivered to the heart and lung. Thus, with traditional techniques (uniform scanning/passive scattering), expanders have been a contraindication to proton therapy. A similar issue exists when using photons, as attenuation of beam creates a cold spot. However, the opposing tangent can compensate for this. Similarly with PBS and use of multifield optimization, the cold spot behind the port can be filled and a similar dose distribution can be created. This technique is currently in use at several facilities in the United States and clinical reports with this technique are awaited.
In general, range uncertainty mitigation includes applying an additional distal margin to the target tissues (CTV). In New Jersey, this consists of 2.5% of the range +2 mm and a smear radius of 7 mm; however, there is some variability between centers regarding these values (88,89). Planners will then generate a series of potential scenarios of range and set-up errors for evaluation and review these to ensure that the nominal plan that still delivers the intended dose distribution assuming uncertainties are maximized. By generating over and under ranged plans while still respecting organ at risk constraints, planners are able to evaluate the “robustness” of a plan, or the certainty that the planned dose delivery matches the actual dose delivery accounting for uncertainties and setup error. An example of a robustness analysis for a patient planned to undergo PMRT with PBS is shown in Figure 4. As described by Depauw et al. (87), coverage of targets and dose to OARs were found to be clinically acceptable, even in the worst case scenario.
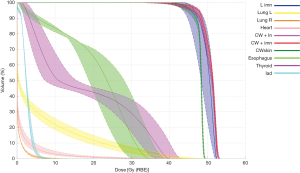
While target motion is typically a problem in the delivery of proton therapy, the targeting of the breast or chest wall and regional lymphatics is spared from dramatic effects because the motion of the target is parallel to the beam path. Due to the inverse square law, minimal changes in the position of the target in the beam axis (as would be expected with chest wall expansion and contraction from respiration) have very little impact on overall dosimetry. This was demonstrated in the treatment planning study published by Ares et al. (53). The investigators reported the change in V95 or V107 as a result of motion for five breast conservation patients planned to undergo proton therapy. Changes in both parameters were small, with average changes below ±1%. The authors concluded that that the problems of motion can probably be ignored. A caveat to the conclusions of this study was that the proton plans were generated for PBS, which is the most sensitive proton modality to motion based on interplay effects. Depauw et al. (87) showed a similar finding with a robustness analysis relative to breathing motion, shown in Figure 5. Compared to the set up uncertainty robustness analysis shown in Figure 4, the worst case scenarios for breathing motion were significantly smaller, further supporting the notion that respiratory motion effects are of no clinical significance.
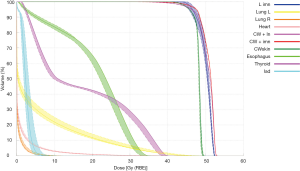
Conclusions and future directions
The ability to comprehensively target tissue at risk while simultaneously sparing normal tissue is an overarching goal of radiation therapy. Multiple dosimetric studies have demonstrated that proton therapy offers a potential ideal balance of coverage and toxicity for patients with breast cancer who require comprehensive nodal treatment in cases of locally advanced disease. Early clinical results with proton therapy are limited but have been encouraging. Mature followup from single arm prospective studies and the RADCOMP study are needed to better define the clinical benefits of proton therapy. Proton therapy has also effectively been used for accelerated PBI for early staged disease, however, whether protons offer an advantage over other forms of PBI needs further study.
Simultaneously, basic, clinical, and translational research continues to refine the role of radiation therapy in general for the treatment of breast cancer. As the best possible applications of this dynamic modality evolve, so too should the use of proton therapy evolve in order to provide the best care to our patients.
Acknowledgements
None.
Footnote
Conflicts of Interest: The authors have no conflicts of interest to declare.
References
- Siegel RL, Miller KD, Jemal A. Cancer statistics, 2015. CA Cancer J Clin 2015;65:5-29. [Crossref] [PubMed]
- Fisher B, Anderson S, Bryant J, et al. Twenty-year follow-up of a randomized trial comparing total mastectomy, lumpectomy, and lumpectomy plus irradiation for the treatment of invasive breast cancer. N Engl J Med 2002;347:1233-41. [Crossref] [PubMed]
- Clarke M, Collins R, Darby S, et al. Effects of radiotherapy and of differences in the extent of surgery for early breast cancer on local recurrence and 15-year survival: an overview of the randomised trials. Lancet 2005;366:2087-106. [Crossref] [PubMed]
- Early Breast Cancer Trialists' Collaborative Group (EBCTCG), Bentzen SM, Agrawal RK, et al. Effect of radiotherapy after breast-conserving surgery on 10-year recurrence and 15-year breast cancer death: meta-analysis of individual patient data for 10,801 women in 17 randomised trials. Lancet 2011;378:1707-16. [Crossref] [PubMed]
- Liljegren G, Holmberg L, Bergh J, et al. 10-Year results after sector resection with or without postoperative radiotherapy for stage I breast cancer: a randomized trial. J Clin Oncol 1999;17:2326-33. [PubMed]
- Veronesi U, Marubini E, Mariani L, et al. Radiotherapy after breast-conserving surgery in small breast carcinoma: long-term results of a randomized trial. Ann Oncol 2001;12:997-1003. [Crossref] [PubMed]
- Douglas BG, Castro JR. Novel fractionation schemes and high linear energy transfer. Prog Exp Tumor Res 1984;28:152-65. [PubMed]
- START Trialists' Group, Bentzen SM, Agrawal RK, et al. The UK Standardisation of Breast Radiotherapy (START) Trial A of radiotherapy hypofractionation for treatment of early breast cancer: a randomised trial. Lancet Oncol 2008;9:331-41. [Crossref] [PubMed]
- Julian TB, Costantino JP, Vicini FA, et al. Early toxicity results with 3D conformal external beam therapy (CEBT) from the NSABP B-39/RTOG 0413 accelerated partial breast irradiation (APBI) trial. J Clin Oncol 2011;29:abstr 1011.
- Formenti SC, Rosenstein B, Skinner KA, et al. T1 stage breast cancer: adjuvant hypofractionated conformal radiation therapy to tumor bed in selected postmenopausal breast cancer patients--pilot feasibility study. Radiology 2002;222:171-8. [Crossref] [PubMed]
- Truong MT, Hirsch AE, Formenti SC. Novel approaches to postoperative radiation therapy as part of breast-conserving therapy for early-stage breast cancer. Clin Breast Cancer 2003;4:253-63. [Crossref] [PubMed]
- Baglan KL, Sharpe MB, Jaffray D, et al. Accelerated partial breast irradiation using 3D conformal radiation therapy (3D-CRT). Int J Radiat Oncol Biol Phys 2003;55:302-11. [Crossref] [PubMed]
- Vicini FA, Remouchamps V, Wallace M, et al. Ongoing clinical experience utilizing 3D conformal external beam radiotherapy to deliver partial-breast irradiation in patients with early-stage breast cancer treated with breast-conserving therapy. Int J Radiat Oncol Biol Phys 2003;57:1247-53. [Crossref] [PubMed]
- Vicini FA, Chen P, Wallace M, et al. Interim cosmetic results and toxicity using 3D conformal external beam radiotherapy to deliver accelerated partial breast irradiation in patients with early-stage breast cancer treated with breast-conserving therapy. Int J Radiat Oncol Biol Phys 2007;69:1124-30. [Crossref] [PubMed]
- Smith BD, Arthur DW, Buchholz TA, et al. Accelerated partial breast irradiation consensus statement from the American Society for Radiation Oncology (ASTRO). Int J Radiat Oncol Biol Phys 2009;74:987-1001. [Crossref] [PubMed]
- Chen PY, Wallace M, Mitchell C, et al. Four-year efficacy, cosmesis, and toxicity using three-dimensional conformal external beam radiation therapy to deliver accelerated partial breast irradiation. Int J Radiat Oncol Biol Phys 2010;76:991-7. [Crossref] [PubMed]
- Lewin AA, Derhagopian R, Saigal K, et al. Accelerated partial breast irradiation is safe and effective using intensity-modulated radiation therapy in selected early-stage breast cancer. Int J Radiat Oncol Biol Phys 2012;82:2104-10. [Crossref] [PubMed]
- Lei RY, Leonard CE, Howell KT, et al. Four-year clinical update from a prospective trial of accelerated partial breast intensity-modulated radiotherapy (APBIMRT). Breast Cancer Res Treat 2013;140:119-33. [Crossref] [PubMed]
- Bergom C, Prior P, Kainz K, et al. A phase I/II study piloting accelerated partial breast irradiation using CT-guided intensity modulated radiation therapy in the prone position. Radiother Oncol 2013;108:215-9. [Crossref] [PubMed]
- Jagsi R, Ben-David MA, Moran JM, et al. Unacceptable cosmesis in a protocol investigating intensity-modulated radiotherapy with active breathing control for accelerated partial-breast irradiation. Int J Radiat Oncol Biol Phys 2010;76:71-8. [Crossref] [PubMed]
- Olivotto IA, Whelan TJ, Parpia S, et al. Interim cosmetic and toxicity results from RAPID: a randomized trial of accelerated partial breast irradiation using three-dimensional conformal external beam radiation therapy. J Clin Oncol 2013;31:4038-45. [Crossref] [PubMed]
- Liss AL, Ben-David MA, Jagsi R, et al. Decline of cosmetic outcomes following accelerated partial breast irradiation using intensity modulated radiation therapy: results of a single-institution prospective clinical trial. Int J Radiat Oncol Biol Phys 2014;89:96-102. [Crossref] [PubMed]
- Hepel JT, Tokita M, MacAusland SG, et al. Toxicity of three-dimensional conformal radiotherapy for accelerated partial breast irradiation. Int J Radiat Oncol Biol Phys 2009;75:1290-6. [Crossref] [PubMed]
- Leonard KL, Hepel JT, Hiatt JR, et al. The effect of dose-volume parameters and interfraction interval on cosmetic outcome and toxicity after 3-dimensional conformal accelerated partial breast irradiation. Int J Radiat Oncol Biol Phys 2013;85:623-9. [Crossref] [PubMed]
- Cahlon O, Ho AY, Siu CT, et al. A Phase II Study of Once a Day Partial Breast Irradiation (PBI) for Early-Stage or Noninvasive Breast Cancer: Early Toxicity Outcomes. Int J Radiat Oncol Biol Phys 2015;93:E15. [Crossref]
- Nichols EM, Feigenberg SJ, Marter K, et al. Preoperative radiation therapy significantly increases patient eligibility for accelerated partial breast irradiation using 3D-conformal radiotherapy. Am J Clin Oncol 2013;36:232-8. [Crossref] [PubMed]
- Horton JK, Blitzblau RC, Yoo S, et al. Preoperative Single-Fraction Partial Breast Radiation Therapy: A Novel Phase 1, Dose-Escalation Protocol With Radiation Response Biomarkers. Int J Radiat Oncol Biol Phys 2015;92:846-55. [Crossref] [PubMed]
- Ödén J, Toma-Dasu I, Yu CX, et al. Dosimetric comparison between intra-cavitary breast brachytherapy techniques for accelerated partial breast irradiation and a novel stereotactic radiotherapy device for breast cancer: GammaPod. Phys Med Biol 2013;58:4409-21. [Crossref] [PubMed]
- Mutaf YD, Zhang J, Yu CX, et al. Dosimetric and geometric evaluation of a novel stereotactic radiotherapy device for breast cancer: the GammaPod. Med Phys 2013;40:041722. [Crossref] [PubMed]
- Formenti SC, Truong MT, Goldberg JD, et al. Prone accelerated partial breast irradiation after breast-conserving surgery: preliminary clinical results and dose-volume histogram analysis. Int J Radiat Oncol Biol Phys 2004;60:493-504. [Crossref] [PubMed]
- Taghian AG, Kozak KR, Katz A, et al. Accelerated partial breast irradiation using proton beams: Initial dosimetric experience. Int J Radiat Oncol Biol Phys 2006;65:1404-10. [Crossref] [PubMed]
- Brashears JH, Dragun AE, Jenrette JM. Late chest wall toxicity after MammoSite breast brachytherapy. Brachytherapy 2009;8:19-25. [Crossref] [PubMed]
- Vargo JA, Verma V, Kim H, et al. Extended (5-year) outcomes of accelerated partial breast irradiation using MammoSite balloon brachytherapy: patterns of failure, patient selection, and dosimetric correlates for late toxicity. Int J Radiat Oncol Biol Phys 2014;88:285-91. [Crossref] [PubMed]
- Weed DW, Edmundson GK, Vicini FA, et al. Accelerated partial breast irradiation: a dosimetric comparison of three different techniques. Brachytherapy 2005;4:121-9. [Crossref] [PubMed]
- Moon SH, Shin KH, Kim TH, et al. Dosimetric comparison of four different external beam partial breast irradiation techniques: three-dimensional conformal radiotherapy, intensity-modulated radiotherapy, helical tomotherapy, and proton beam therapy. Radiother Oncol 2009;90:66-73. [Crossref] [PubMed]
- Kozak KR, Smith BL, Adams J, et al. Accelerated partial-breast irradiation using proton beams: initial clinical experience. Int J Radiat Oncol Biol Phys 2006;66:691-8. [Crossref] [PubMed]
- Galland-Girodet S, Pashtan I, MacDonald SM, et al. Long-term cosmetic outcomes and toxicities of proton beam therapy compared with photon-based 3-dimensional conformal accelerated partial-breast irradiation: a phase 1 trial. Int J Radiat Oncol Biol Phys 2014;90:493-500. [Crossref] [PubMed]
- Chang JH, Lee NK, Kim JY, et al. Phase II trial of proton beam accelerated partial breast irradiation in breast cancer. Radiother Oncol 2013;108:209-14. [Crossref] [PubMed]
- Bush DA, Slater JD, Garberoglio C, et al. Partial breast irradiation delivered with proton beam: results of a phase II trial. Clin Breast Cancer 2011;11:241-5. [Crossref] [PubMed]
- Bush DA, Do S, Lum S, et al. Partial breast radiation therapy with proton beam: 5-year results with cosmetic outcomes. Int J Radiat Oncol Biol Phys 2014;90:501-5. [Crossref] [PubMed]
- Overgaard M, Hansen PS, Overgaard J, et al. Postoperative radiotherapy in high-risk premenopausal women with breast cancer who receive adjuvant chemotherapy. Danish Breast Cancer Cooperative Group 82b Trial. N Engl J Med 1997;337:949-55. [Crossref] [PubMed]
- Overgaard M, Jensen MB, Overgaard J, et al. Postoperative radiotherapy in high-risk postmenopausal breast-cancer patients given adjuvant tamoxifen: Danish Breast Cancer Cooperative Group DBCG 82c randomised trial. Lancet 1999;353:1641-8. [Crossref] [PubMed]
- Ragaz J, Olivotto IA, Spinelli JJ, et al. Locoregional radiation therapy in patients with high-risk breast cancer receiving adjuvant chemotherapy: 20-year results of the British Columbia randomized trial. J Natl Cancer Inst 2005;97:116-26. [Crossref] [PubMed]
- Whelan TJ, Olivotto IA, Parulekar WR, et al. Regional Nodal Irradiation in Early-Stage Breast Cancer. N Engl J Med 2015;373:307-16. [Crossref] [PubMed]
- Poortmans PM, Collette S, Kirkove C, et al. Internal Mammary and Medial Supraclavicular Irradiation in Breast Cancer. N Engl J Med 2015;373:317-27. [Crossref] [PubMed]
- Harris EE, Correa C, Hwang WT, et al. Late cardiac mortality and morbidity in early-stage breast cancer patients after breast-conservation treatment. J Clin Oncol 2006;24:4100-6. [Crossref] [PubMed]
- Correa CR, Litt HI, Hwang WT, et al. Coronary artery findings after left-sided compared with right-sided radiation treatment for early-stage breast cancer. J Clin Oncol 2007;25:3031-7. [Crossref] [PubMed]
- Nilsson G, Holmberg L, Garmo H, et al. Distribution of coronary artery stenosis after radiation for breast cancer. J Clin Oncol 2012;30:380-6. [Crossref] [PubMed]
- Darby SC, Ewertz M, McGale P, et al. Risk of ischemic heart disease in women after radiotherapy for breast cancer. N Engl J Med 2013;368:987-98. [Crossref] [PubMed]
- Arthur DW, Arnfield MR, Warwicke LA, et al. Internal mammary node coverage: an investigation of presently accepted techniques. Int J Radiat Oncol Biol Phys 2000;48:139-46. [Crossref] [PubMed]
- Jagsi R, Moran J, Marsh R, et al. Evaluation of four techniques using intensity-modulated radiation therapy for comprehensive locoregional irradiation of breast cancer. Int J Radiat Oncol Biol Phys 2010;78:1594-603. [Crossref] [PubMed]
- Fontanilla HP, Woodward WA, Lindberg ME, et al. Current clinical coverage of Radiation Therapy Oncology Group-defined target volumes for postmastectomy radiation therapy. Pract Radiat Oncol 2012;2:201-9. [Crossref] [PubMed]
- Ares C, Khan S, Macartain AM, et al. Postoperative proton radiotherapy for localized and locoregional breast cancer: potential for clinically relevant improvements? Int J Radiat Oncol Biol Phys 2010;76:685-97. [Crossref] [PubMed]
- Fagundes MA, Pankuch M, Hartsell W, et al. Cardiac-sparing postmastectomy proton radiation therapy for women with stage III, loco-regional breast cancer: a dosimetric comparison study. Int J Radiat Oncol Biol Phys 2013;87:S245. [Crossref]
- MacDonald SM, Jimenez R, Paetzold P, et al. Proton radiotherapy for chest wall and regional lymphatic radiation; dose comparisons and treatment delivery. Radiat Oncol 2013;8:71. [Crossref] [PubMed]
- MacDonald SM, Patel SA, Hickey S, et al. Proton therapy for breast cancer after mastectomy: early outcomes of a prospective clinical trial. Int J Radiat Oncol Biol Phys 2013;86:484-90. [Crossref] [PubMed]
- Mast ME, Vredeveld EJ, Credoe HM, et al. Whole breast proton irradiation for maximal reduction of heart dose in breast cancer patients. Breast Cancer Res Treat 2014;148:33-9. [Crossref] [PubMed]
- Xu N, Ho MW, Li Z, et al. Can proton therapy improve the therapeutic ratio in breast cancer patients at risk for nodal disease? Am J Clin Oncol 2014;37:568-74. [Crossref] [PubMed]
- Bradley JA, Dagan R, Ho MW, et al. Initial Report of a Prospective Dosimetric and Clinical Feasibility Trial Demonstrates the Potential of Protons to Increase the Therapeutic Ratio in Breast Cancer Compared With Photons. Int J Radiat Oncol Biol Phys 2016;95:411-21. [Crossref] [PubMed]
- Cuaron JJ, Chon B, Tsai H, et al. Early toxicity in patients treated with postoperative proton therapy for locally advanced breast cancer. Int J Radiat Oncol Biol Phys 2015;92:284-91. [Crossref] [PubMed]
- Cuaron JJ, Cheng C, Joseph H, et al. Dosimetric Comparison of Skin Surface Dose in Patients Undergoing Proton and Photon Radiation Therapy for Breast Cancer. Int J Radiat Oncol Biol Phys 2014;90:S914.
- Kochanek KD, Murphy SL, Xu J. Deaths: Final Data for 2011. Natl Vital Stat Rep 2015;63:1-120. [PubMed]
- Tian S, Hirshfield KM, Jabbour SK, et al. Serum biomarkers for the detection of cardiac toxicity after chemotherapy and radiation therapy in breast cancer patients. Front Oncol 2014;4:277. [Crossref] [PubMed]
- Sawaya H, Sebag IA, Plana JC, et al. Assessment of echocardiography and biomarkers for the extended prediction of cardiotoxicity in patients treated with anthracyclines, taxanes, and trastuzumab. Circ Cardiovasc Imaging 2012;5:596-603. [Crossref] [PubMed]
- Erven K, Florian A, Slagmolen P, et al. Subclinical cardiotoxicity detected by strain rate imaging up to 14 months after breast radiation therapy. Int J Radiat Oncol Biol Phys 2013;85:1172-8. [Crossref] [PubMed]
- Tan T, Chin J, Lu H, et al. Assessment of cardiac function following proton radiation in a cohort of post mastectomy patients with locally advanced breast cancer. J Am Coll Cardiol 2015.65.
- Marks LB, Yu X, Prosnitz RG, et al. The incidence and functional consequences of RT-associated cardiac perfusion defects. Int J Radiat Oncol Biol Phys 2005;63:214-23. [Crossref] [PubMed]
- Prosnitz RG, Hubbs JL, Evans ES, et al. Prospective assessment of radiotherapy-associated cardiac toxicity in breast cancer patients: analysis of data 3 to 6 years after treatment. Cancer 2007;110:1840-50. [Crossref] [PubMed]
- Heggemann F, Grotz H, Welzel G, et al. Cardiac Function After Multimodal Breast Cancer Therapy Assessed With Functional Magnetic Resonance Imaging and Echocardiography Imaging. Int J Radiat Oncol Biol Phys 2015;93:836-44. [Crossref] [PubMed]
- Chen T, Reyhan M, Yue N, et al. Tagged MRI based cardiac motion modeling and toxicity evaluation in breast cancer radiotherapy. Front Oncol 2015;5:9. [Crossref] [PubMed]
- White J, Tai A, Arthur D, et al. Breast Cancer Atlas for Radiation Therapy Planning: Consensus Definitions. Available online: https://www.rtog.org/LinkClick.aspx?fileticket=vzJFhPaBipE%3d&tabid=236
- Reed VK, Cavalcanti JL, Strom EA, et al. Risk of subclinical micrometastatic disease in the supraclavicular nodal bed according to the anatomic distribution in patients with advanced breast cancer. Int J Radiat Oncol Biol Phys 2008;71:435-40. [Crossref] [PubMed]
- Vargo JA, Beriwal, S. Are the current RTOG Contouring Atlas definitions for supraclavicular lymph nodes adequate for all breast cancer patients? Available online: http://appliedradiationoncology.com/articles/are-the-current-rtog-contouring-atlas-definitions-for-supraclavicular-lymph-nodes-adequate-for-all-breast-cancer-patients
- Cuaron JJ, Hug E, Chon BH, et al. Coverage of Posterior Supraclavicular Fossa With Postmastectomy Proton Therapy for Breast Cancer: A Dosimetric Comparison Study and Implications for Target Definition. Int J Radiat Oncol Biol Phys 2014;90:S914.
- Paganetti H, Niemierko A, Ancukiewicz M, et al. Relative biological effectiveness (RBE) values for proton beam therapy. Int J Radiat Oncol Biol Phys 2002;53:407-21. [Crossref] [PubMed]
- Paganetti H. Relative biological effectiveness (RBE) values for proton beam therapy. Variations as a function of biological endpoint, dose, and linear energy transfer. Phys Med Biol 2014;59:R419-72. [Crossref] [PubMed]
- Robertson JB, Williams JR, Schmidt RA, et al. Radiobiological studies of a high-energy modulated proton beam utilizing cultured mammalian cells. Cancer 1975;35:1664-77. [Crossref] [PubMed]
- Tepper J, Verhey L, Goitein M, et al. In vivo determinations of RBE in a high energy modulated proton beam using normal tissue reactions and fractionated dose schedules. Int J Radiat Oncol Biol Phys 1977;2:1115-22. [Crossref] [PubMed]
- Hall EJ, Kellerer AM, Rossi HH, et al. The relative biological effectiveness of 160 MeV protons--II. Biological data and their interpretation in terms of microdosimetry. Int J Radiat Oncol Biol Phys 1978;4:1009-13. [Crossref] [PubMed]
- Raju MR, Amols HI, Bain E, et al. A heavy particle comparative study. Part III: OER and RBE. Br J Radiol 1978;51:712-9. [Crossref] [PubMed]
- Urano M, Goitein M, Verhey L, et al. Relative biological effectiveness of a high energy modulated proton beam using a spontaneous murine tumor in vivo. Int J Radiat Oncol Biol Phys 1980;6:1187-93. [Crossref] [PubMed]
- Gerweck LE, Kozin SV. Relative biological effectiveness of proton beams in clinical therapy. Radiother Oncol 1999;50:135-42. [Crossref] [PubMed]
- Britten RA, Nazaryan V, Davis LK, et al. Variations in the RBE for cell killing along the depth-dose profile of a modulated proton therapy beam. Radiat Res 2013;179:21-8. [Crossref] [PubMed]
- Matsumoto Y, Matsuura T, Wada M, et al. Enhanced radiobiological effects at the distal end of a clinical proton beam: in vitro study. J Radiat Res 2014;55:816-22. [Crossref] [PubMed]
- Chaudhary P, Marshall TI, Perozziello FM, et al. Relative biological effectiveness variation along monoenergetic and modulated Bragg peaks of a 62-MeV therapeutic proton beam: a preclinical assessment. Int J Radiat Oncol Biol Phys 2014;90:27-35. [Crossref] [PubMed]
- Cuaron JJ, Chang C, Lovelock M, et al. Exponential Increase in Relative Biological Effectiveness Along Distal Edge of a Proton Bragg Peak as Measured by Deoxyribonucleic Acid Double-Strand Breaks. Int J Radiat Oncol Biol Phys 2016;95:62-9. [Crossref] [PubMed]
- Depauw N, Batin E, Daartz J, et al. A novel approach to postmastectomy radiation therapy using scanned proton beams. Int J Radiat Oncol Biol Phys 2015;91:427-34. [Crossref] [PubMed]
- Moyers MF, Miller DW, Bush DA, et al. Methodologies and tools for proton beam design for lung tumors. Int J Radiat Oncol Biol Phys 2001;49:1429-38. [Crossref] [PubMed]
- Paganetti H. Range uncertainties in proton therapy and the role of Monte Carlo simulations. Phys Med Biol 2012;57:R99-117. [Crossref] [PubMed]